Learning Objectives
- Differentiate between the general types of cell signals (autocrine, endocrine, etc.) and classes of hormones (polypeptide, amino acid, and steroid)
- Define signal transduction and describe the general pathways of signal transduction and amplification for steroid vs non-steroid hormones
- Define and differentiate between positive and negative feedback loops in hormonal control
- Define and recognize crosstalk and other sources of complexity in signaling such as different receptors and/or different signaling pathways in different cell types
- Define quorum sensing and describe the role of cell signaling microbe behaviors
Communication Between and Within Cells
The information below was adapted from OpenStax Biology 9.1 and Khan Academy Introduction to cell signaling. All Khan Academy content is available for free at www.khanacademy.org
Cells can detect what’s going on around them, and they can respond in real time to cues from their neighbors and environment. At this very moment, your cells are sending and receiving millions of messages in the form of chemical signaling molecules. There are two general kinds of cellular chemical communication:
- communication between cells is called intercellular signaling (“inter-” in Latin means “between”; like the way interstates allow rapid travel between states
- communication within a cell is called intracellular signaling (“intra-” in Latin means “inside”; like intravenous is within the veins
Chemical signals sent between cells are called ligands. A ligand is a molecule that binds another specific molecule. In the case of cell signaling, the ligand binds a receptor, which is a protein in or on the target cell (more on that distinction later). Examples of ligands include hormones and neurotransmitters. Specificity in cell signaling occurs in a couple different ways:
- Ligands and receptors are highly specific in the ways they binding to each other: each receptor is typically only able to bind to one very specific ligand
- Not all cells have receptors for each ligand, meaning that only cells that have the receptor are able to detect or respond to a particular ligand
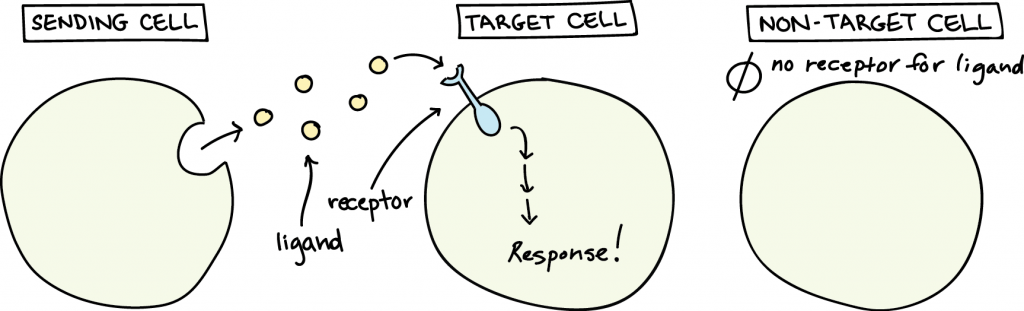
Categories of Cell Signaling
How does a particular ligand reach a particular cell? There are five categories of chemical signaling found in multicellular organisms:
- Direct signaling (also called juxtacrine signaling) involves communication between cells that are in direct contact with each other. This communication is often mediated by gap junctions in animal cells and plasmodesmata in plant cells.
- Autocrine signaling occurs when a ligand acts on the same cell that releases it.
- Paracrine signaling occurs when a ligand diffuses in a small area and only acts on neighboring cells. Neural signals are a specialized subset of paracrine signals, diffusing a very short distance within the synaptic cleft between adjacent neurons (more on neural communication in a future class session).
- Endocrine signals are hormones, which are carried throughout the organism’s body via the vascular system (in either plants or animals!) to act on cells that may be very far away from the cells which released the ligand (more on plant and animal hormones in future class sessions).
- Neuroendocrine signals are a specialized subcategory of endocrine signals that are released by neurons but travel via the vascular system to act on cells that are far away.
- Pheromones are released into the environment to act on the cells in a different individual.
Each of these types of signaling are briefly described below.

The video below provides a concise overview of a few of these processes above:
Functions and Types of Hormones
The information below was adapted from OpenStax Biology 37.2, and OpenStax Biology 9.2
The rest of this reading focuses on chemical signaling via hormones (endocrine signaling). Hormones regulate many different functions, including homeostasis, development, reproduction, and stress. Though we tend to think of them as associated with animals, hormones function in cell signaling in all multicellular organisms and, arguably, even in single-celled organisms (more on that at the end of this reading).
Hormones are defined by shared function rather than shared structure. A molecule is a hormone if it:
- is secreted from a cell or gland into the vascular system (or into the environment, in the case of single-celled organisms)
- acts on distant cells in other locations in the body (or community, in the case of single-celled organisms); this phenomenon occurs because the hormone travels through the circulatory system (or environment)
- causes large effects even with only small amounts of the molecule; this phenomenon occurs due to signal amplification
- causes a response only in specific target cells even though they come into contact with many different cell types; this phenomenon occurs because only those specific target cells possess a receptor that is capable of binding to that particular hormone
- causes a characteristic response (always the same response in a given set of circumstances); this phenomenon occurs because activation of the receptor by the hormone initiates a specific signaling pathway in response to the hormone
- is part of a feedback loop (either positive or negative)
There are four general structural classes of hormones, which often dictates how these hormones interact with a cell’s receptor:
- Hydrophilic hormones are soluble in water or aqueous solution, meaning that they cannot cross the cell’s hydrophobic plasma membrane. The receptor for a hydrophilic hormone must be present on the cell’s surface for the hormone to reach it. Hydrophilic hormones tend to include:
- peptide hormones, which are small proteins
- amino acid-derived hormones, which are modified amino acids (the building blocks of proteins)
- Hydrophobic hormones are typically small nonpolar molecules that are insoluble in water, meaning that they can easily cross the cell’s hydrophobic plasma membrane. The receptor for a hydrophobic hormone is located within a cell’s cytoplasm or nucleus. Hydrophobic hormones tend to include:
- steroid hormones, which are small organic compounds with characteristic carbon ring structures
- gas hormones, which are gases capable of acting like ligands
Hormone Signaling Pathways and Steps
The information below was adapted from OpenStax Biology 9.2 and Khan Academy Ligands & receptors. All Khan Academy content is available for free at www.khanacademy.org
Receiving and responding to a hormone signal typically involves an ordered of characteristic steps:
Step 1: signal reception. The first step in hormone signaling is the hormone binding to the receptor. This can occur either inside the cell or on the cell surface, depending on the class of hormone.
- Nonpolar, hydrophobic ligands (such as steroid and gas hormones) that are able to travel across the plasma membrane bind to internal receptors, also known as intracellular or cytoplasmic receptors, found in the cytoplasm of the cell.
- Once the hormone binds, the receptor changes shape, allowing the receptor-hormone complex to enter the nucleus (if it wasn’t there already).
- The hormone-receptor complex is then capable of binding to specific sequences of DNA and activating or repressing transcription of certain genes (the receptor alone can’t do this; it requires the hormone to bind to the DNA).
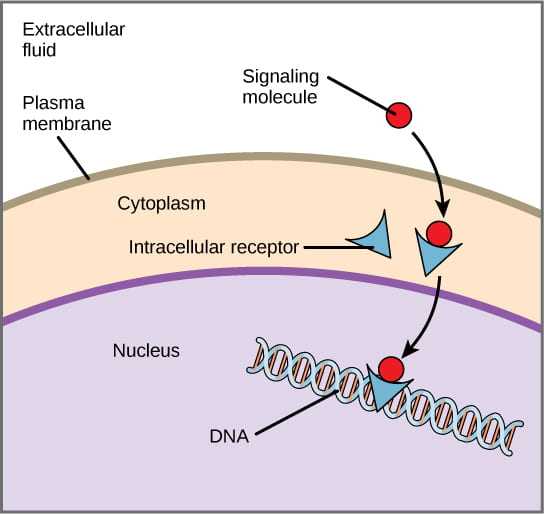
- Hydrophilic ligands (such as peptide and amino acid-derived hormones) cannot cross the cell plasma membrane and must bind to cell-surface receptors, also known as transmembrane receptors, on the cell surface.
- Once the hormone binds, the receptor changes shape and activates chemical or protein “messengers” to relay the signal from outside the cell to inside the cell
- This signal relay process is called signal transduction, which is the process of converting a signal from one form to another. In the case of hormone signaling, signal transduction means converting an extracellular signal into an intracellular signal.
- As a result, the receptor does not alter gene expression directly, but must activate chemical or protein “messengers” to relay the signal from outside to inside the cell.
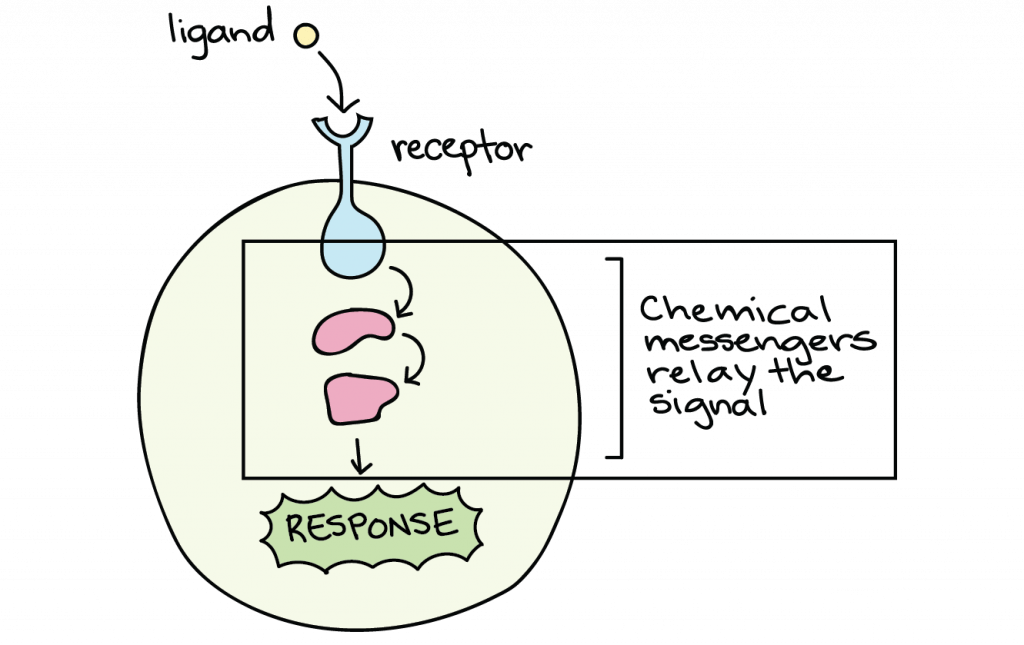
Step 2: signal transduction. This step is necessary only for hydrophilic ligands that must bind extracellular receptors because they cannot cross the plasma membrane.
- Once a hormone binds to the extracellular portion of the cell-surface receptor, the intracellular portion of the receptor changes shape, resulting in activation of a chain of events that is called a signaling pathway or signaling cascade.
- The events in the cascade occur in a defined series of events. Many different enzymes are activated by different specific receptors, but in general this activated enzyme then activates other proteins which carry the signal into the cell to elicit a response.
- Pathways activated by cell surface receptors might include either:
- synthesis of second messengers (non-protein signaling molecules) such as calcium or cyclic AMP which propagate throughout the cell to spread the signal, or
- initiation of a phosphorylation cascade where a series of proteins are activated by having a phosphate group added to them, which changes their activity.
- Ultimately the activation of the pathway results in some type of cellular response, which may include changes in gene expression.
Step 3: signal amplification: one feature of hormones is that only a very small amount of the hormone can result in a very strong physiological response. This phenomenon is mediated through a process called signal amplification, where the signal from the hormone is “amplified” or magnified via one of several mechanisms:
- For hydrophilic hormones that bind to cell-surface receptors, the amplification can occur via second messengers, where thousands of molecules are produced or released in response to the hormone signal, or through or phosphorylation cascades, where thousands of proteins become activated
- For nonpolar hormones that bind to intracellular receptors, the amplification can occur during the process of both transcription, where hundreds of copies of mRNA are synthesized from a single gene, and during translation, where hundreds of copies of each protein are synthesized from a single mRNA
The video below provides an overview receptor-ligand binding, signal transduction, and a preview cellular responses (covered in the next section):
The information below was adapted from OpenStax Biology 9.3, and Khan Academy Signal relay pathways. All Khan Academy content is available for free at www.khanacademy.org
Signal Response and Feedback Loops
The last stage of a signaling pathway is the signal response. There are many different types of cellular responses to a hormone, including changes in gene expression, changes in cell metabolism, and/or changes in cell growth and division. These responses are often carried out in one of two types of feedback loops:
- a positive feedback loop occurs when the response to a hormone causes the original signal to be amplified or increase. Think of a rock concert where the musicians turn up the music causing the crowd to be more excited; the excitement of the crowd causes the musicians to play louder, further increasing the excitement in the crowd. The signal in this scenario has increased in a positive feedback loop.
- a negative feedback loop occurs when the response to a hormone causes the original signal to be decreased. Think of a thermostat where the temperature in the room becomes too hot; the heat of the room causes the thermostat to activate the air conditioning, and the temperature in the room becomes cooler. The signal in this scenario has decreased in a negative feedback loop.
This video (beginning at 1:13) provides an overview of positive vs negative feedback loops (watch through at least 5:58):
Signaling Crosstalk and other Complexities
The response to a particular signal can be very straightforward, but there can be differences between different cell types and in different conditions for a number of reasons:
- In some cases, a single hormone can act on several different cell types causing a different response in each because the cells themselves carry out different functions and have different intracellular proteins that respond to the hormone.
- For example, the hormone adrenaline causes liver cells to release glucose into the blood stream, and it causes blood vessels in skeletal muscle to dilate. These different responses occur even though both cell types have the same type of receptor to bind to adrenaline.
- In other cases, the same hormone can cause different responses in different cells due to different receptors in the two different cell types, which then activate different signaling pathways and ultimately leading to different responses in each cell type.
- For example, adrenaline causes blood vessels in skeletal muscle to dilate, but it causes blood vessels in the intestine to constrict. These different responses occur because blood vessels in skeletal muscle have a different type of adrenaline receptor compared to blood vessels in the intestines.
- Often multiple signaling pathways interact with each other because the same signaling proteins are involved in multiple pathways. As a result, if signals from two different hormones are received at the same time, the outcome can be different than if they came in separately. The phenomenon where the outcome can change based on interactions between different signaling pathways is called signaling crosstalk.
- For example, when blood glucose levels are high, the hormone insulin causes muscle cells to take up the excess glucose to remove it from the blood. However, when adrenaline is also present, the adrenaline signaling pathway in muscle cells blocks the insulin signaling pathway, meaning that the muscle cells do not take up excess glucose when adrenaline is present, even when insulin is also present.
Signaling in Single-Celled Organisms
The information below was adapted from OpenStax Biology 9.0 and OpenStax Biology 9.4, OpenStax Biology 22.1, Khan Academy Cell-cell signaling in unicellular organisms, and Wikipedia “Quorum sensing”. All Khan Academy content is available for free at www.khanacademy.org
In multicellular organisms, cells are constantly sending and receiving chemical messages to coordinate the actions of distant organs, tissues, and cells.
While the need for cellular communication in larger organisms seems obvious, even single-celled organisms communicate with each other. For example, yeast produce chemical signals that allow them to find mates. Some species of bacteria coordinate their actions in order to form large complexes called biofilms, or to organize the production of toxins to kill competing organisms. The ability of cells to communicate through chemical signals originated in single cells and was essential for the evolution of multicellular organisms.
For the last part of this reading, we will explore the principles and several examples of a phenomenon called quorum sensing, a phenomenon where individual single cells monitor the density of their population; once the population reaches a specific density, the cells collectively change their gene expression and behavior at the same time. Quorum sensing was originally discovered in bacteria, but it has been subsequently identified in many other single-celled organisms.
Quorum sensing is used to control a number of behaviors that would be difficult or even harmful for one or a few bacteria to perform, but which become successful and highly adaptive if performed by a large group at the same time. Because the number of cells present in the environment (cell density) is the determining factor for signaling, this type of signaling is named “quorum” sensing; in politics and business, a quorum is the minimum number of members required to be present to vote on an issue. Behaviors regulated by quorum sensing include (but are not limited to):
- formation of biofilms
- attacking competitors
- luminescence (emitting light)
- activation of virulence genes (associated with pathogenicity, or the ability to cause an infection)
How does quorum sensing work? It’s all based on continuous secretion of a signaling molecule, or a bacterial hormone, called an autoinducer. If a single cell or just a few cells secrete it, there isn’t much of the molecule in environment and it doesn’t change the bacteria’s behavior. But as the number of individuals in the population increases, and there are more and more cells secreting the hormone, the hormone will gradually increase in density. Once it reaches a certain density, it causes a cellular response in all cells at the same time; the cells collectively change their behavior based on whatever signaling pathway is activated by the hormone.

In general, each species of bacteria has its own autoinducer, with a matching receptor that’s highly specific (won’t be activated by the autoinducer of a different bacterium). However, some types of autoinducers can be produced and detected by multiple species of bacteria. Scientists are investigating how these molecules may allow for between-species communication.
We’ll describe a few examples of behaviors controlled by quorum sensing below:
- Symbiosis and bioluminescence: Quorum sensing was first discovered in Aliivibrio fischeri, a bacterium that has a symbiotic (mutually beneficial) relationship with the Hawaiian bobtail squid. A. fischeri form colonies inside the squid’s “light organ.” The squid gives the bacteria food, and in return, the bacteria emit light through a process called bioluminescence. The glow of the bacteria prevents the squid from casting a shadow, hiding it from predators swimming beneath.
- A. fischeri bacteria only glow when they are inside of a squid’s light organ; they don’t glow when they are free-living in the ocean. Through decades of work, scientists discovered that the bacteria use quorum sensing to decide when to produce bioluminescence. It would be a metabolic waste for a lone bacterium in the open ocean to carry out chemical reactions that emit light, since they provide no benefit without a squid host. But when many bacteria are tightly packed in a light organ, the action of glowing in unison provides an advantage: it allows the bacteria to fulfill their end of the symbiotic bargain, keeping their squid host (their food source) from being eaten by predators.
- The autoinducer that controls A. fischeri‘s behavior activates transcription of genes that encode enzymes and substrates required for bioluminescence, as well as the gene for the enzyme that makes the autoinducer itself (amplifying the response in a positive feedback loop).
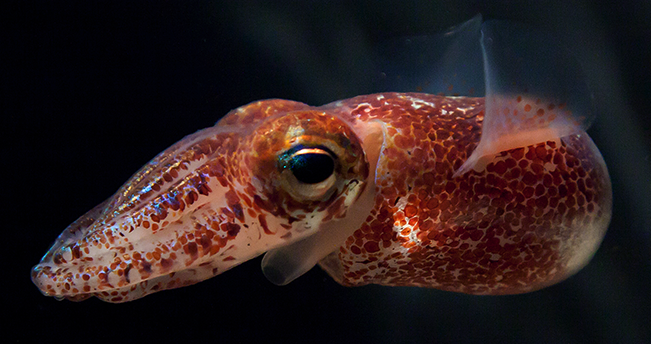
This video describes the behavior of A. fischeri and the Hawaiian bobtail squid:
- Biofilm formation: Some species of quorum-sensing bacteria form biofilms, which are communities of bacterial cells that stick to one another and to a surface. Biofilms can be quite complex, with bacterial cells organizing to form ordered structures, and some biofilms contain multiple species of coexisting bacteria.
- Biofilms are present almost everywhere: they produce dental plaque (if you haven’t brushed your teeth recently, you might feel the start of a biofilm colonizing your teeth), and they colonize catheters, prostheses, transcutaneous and orthopedic devices, contact lenses, and internal devices such as pacemakers. It is estimated that 65 percent of all infections acquired in the hospital are due to biofilms. Biofilms are also related to diseases contracted from food because they colonize the surfaces of vegetable leaves and meat, as well as food-processing equipment that isn’t adequately cleaned.
- Biofilms are more robust and resilient than free-living bacteria. The sticky substance that holds the bacteria together also excludes most antibiotics and disinfectants, so it’s harder to kill the cells in a biofilm. In addition, individual cells within a biofilm actually take on specialized roles, similar to multicellular organisms, with some cells forming channels to help remove toxins (such as antibiotics) from the biofilm.
- Once an infection by a biofilm is established, it is very difficult to eradicate, because biofilms tend to be resistant to most of the methods used to control microbial growth, including antibiotics and detergents. Biofilms respond poorly or only temporarily to antibiotics; it has been said that they can resist up to 1,000 times the antibiotic concentrations used to kill the same bacteria when they are free-living. An antibiotic dose that large would harm the patient; therefore, scientists are working on new ways to get rid of biofilms.
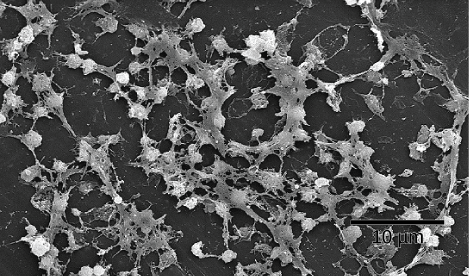
This video provides a quick overview of the relevance of biofilms to human health:
- Virulence: Virulence, or the ability of a microorganism to cause disease, is also controlled by quorum sensing. As one example, the opportunistic pathogen Pseudomonas aeruginosa uses quorum sensing to coordinate the formation an array of behaviors including virulence.
- These bacteria can grow within a host without harming it, until they reach a threshold concentration. Once they have replicated to the point at which their numbers are sufficient to overcome the host’s immune system, quorum sensing induces expression of virulence genes, causing the bacteria to become aggressively infectious and also a biofilm.
- This change in bacterial behavior not only leads to infection and disease within the host, but also prevents the host’s immune system from combating the bacteria as the biofilm provides a protective layer encasing the bacteria population away from the immune cells.
- This type of infection is particularly common in the lungs of patients with cystic fibrosis, and is extremely difficult to treat. Quorum sensing-mediated virulence pathways play a role in infection caused by many other species of bacteria, which infect both animals and plants.
Research on the details of quorum sensing has led to advances in growing bacteria for industrial purposes. Recent discoveries suggest that it may be possible to exploit bacterial signaling pathways to control bacterial growth; this process could replace or supplement antibiotics that are no longer effective in certain situations.