Learning Objectives
- Use the Law of Partial Pressures to predict direction of gas movement in solution
- Explain the functional adaptations present in animal respiratory surfaces in the context of Fick’s Law of Diffusion (surface area, distance, concentration gradients, passage of blood through the respiratory surface)
- Compare and contrast the structure/function of respiratory surfaces including skin, gills, tracheae, avian lungs, and mammalian lungs; and identify and explain why which is/are the most efficient for gas exchange
- Describe how oxygen and carbon dioxide are transported in vertebrate respiratory systems
- Use dissociation curves to describe cooperative and reversible binding of O2 to hemoglobin
- Use dissociation curves to predict the effects of changes in pH, temperature, and CO2 concentrations on hemoglobin affinity for O2
The Law of Partial Pressures and the Basic Principles of Gas Exchange
The information below was adapted from OpenStax Biology 39.2
Eukaryotic cells continually produce carbon dioxide as a metabolic waste product, and continually require oxygen as the terminal electron acceptor in mitochondria for cellular respiration. Multicellular eukaryotes must have a mechanism for eliminating carbon dioxide and taking in oxygen, which occurs via a process called gas exchange. Gas exchange during respiration occurs primarily through diffusion, where the gas molecules move from a region of high concentration to a region of low concentration.
The gasses being exchanged exist within a mixture of other molecules, and each component in the mixture exerts its own partial pressure. Partial pressure is a measure of the concentration of each individual component within the overall mixture of gases. The total pressure exerted by the mixture is the sum of the individual partial pressures of the components in the mixture. This matters for gas exchange because the rate of diffusion of a gas is proportional to its partial pressure within the total gas mixture: a gas with a strong partial pressure gradient (very high partial pressure on one side, very low partial pressure on the other side) will diffuse faster than a gas with a weak partial pressure gradient.
What does partial pressure look like in the air that we breathe? Air is primarily nitrogen (N2; 78.6 percent), oxygen (O2; 20.9 percent), water vapor (H2O; 0.5 percent), and carbon dioxide (CO2; 0.04 percent). Because approximately 21 percent of atmospheric gas is oxygen, and approximately 0.04 percent is carbon dioxide, the partial pressure for oxygen is much greater than that of carbon dioxide.
The partial pressure of any gas can be calculated by: P = (Patm) x (% content in mixture), where Patm, is the atmospheric pressure at any given altitude, calculated as the sum of all of the partial pressures of the atmospheric gases added together. At sea level: Patm = PN2 +PO2+ PH2O+ PCO2= 760 mm Hg
Therefore, the partial pressure of oxygen at sea level (Patm = 760 mm Hg) is: PO2 = (760 mm Hg) (0.21) = 160 mm Hg. And the partial pressure of carbon dioxide at sea level is: PCO2 = (760 mm Hg) (0.0004) = 0.3 mm Hg.
As altitude increases above sea level, the concentration of each gas does not change, but Patm decreases; as a result, partial pressure of each gas decreases as altitude increases.
These pressures determine the gas exchange, or the flow of gas, in the system. Oxygen and carbon dioxide will flow according to their pressure gradient from high to low within the respiratory system.
Fick’s Law of Diffusion: the Rules of Gas Exchange and the Features of Respiratory Surfaces
The information below was adapted from OpenStax Biology 39.2
Gasses move “down” their partial pressure gradient (from areas of high concentration to areas of low concentration. The rate of diffusion of a gas across a surface is controlled by the following:
- k, the gas diffusion constant
- A, the area for gas exchange
- P2-P1, the difference in partial pressure of gas on either side of diffusion barrier
- D, the distance across which the gas must diffuse (thickness of diffusion barrier)
These terms are related by the following equation: Rate of diffusion = k x A x (P2-P1)/D
Effectively this means that gas will diffuse most quickly when the following criteria are met:
- the area (A) available for gas exchange is large
- the difference in partial pressure (P2-P1) is large
- the distance (D) required for gas to move from one area to another is small
What does this mean for respiratory surfaces, like lungs and gills, where gas exchange occurs? Evolution cannot control the partial pressure of gas in the air (or water, for aquatic species), but it has optimized what it can control: evolution has selected for respiratory surfaces with very large surface areas, very thin membranes separating the locations for gas exchange, and using passage of blood to take advantage of the location within the body with the greatest concentration gradient between blood and respiratory surface:
- The structure of any respiratory surface (lungs, gills, tracheae), maximizes its surface area to increase gas diffusion. Because of the enormous number of alveoli (approximately 300 million in each human lung), the surface area of the lung is very large (75 m2). Having such a large surface area increases the amount of gas that can diffuse into and out of the lungs.
- Respiratory surfaces are also extremely thin (typically only one cell thick), minimizing the distance gas must diffuse across the surface.
- Capillaries bring blood to the respiratory surface for gas exchange, where the blood entering the respiratory organ is low in oxygen concentration and high in carbon dioxide concentration; because the air in the lungs has a higher concentration of oxygen than that of oxygen-depleted blood and a lower concentration of carbon dioxide, this concentration gradient allows for gas exchange during respiration.
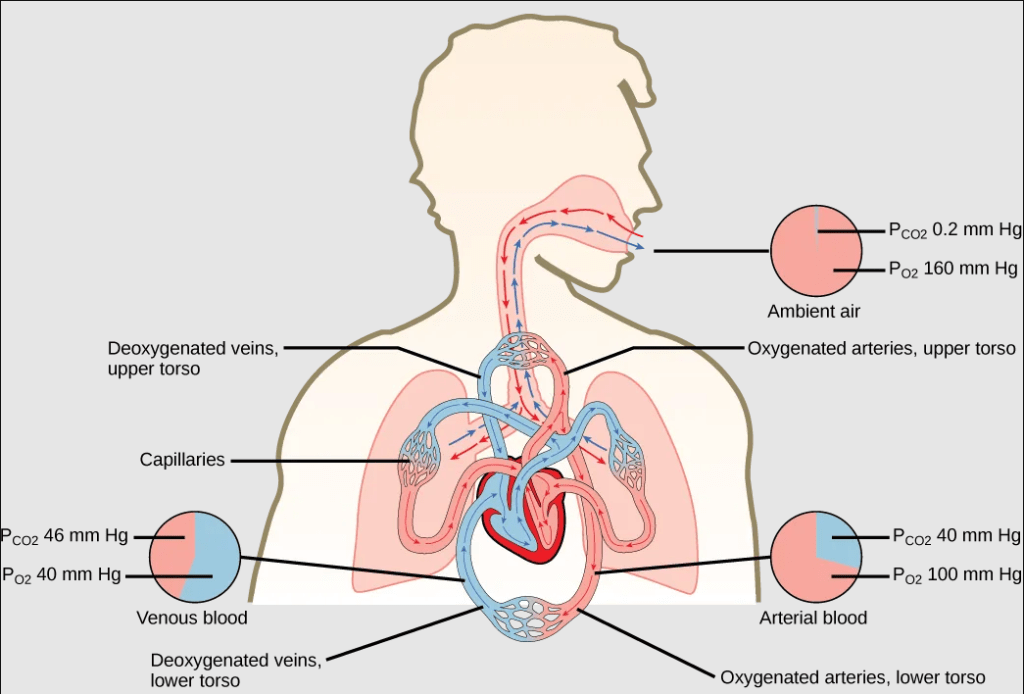
Variation in Respiratory Surfaces
The information below was adapted from OpenStax Biology 39.1 and OpenStax Biology 39.3
Gas exchange in complex organisms typically involved three steps:
- Ventilation: air is brought into the respiratory organ
- Gas exchange: oxygen is taken in, and carbon dioxide is expelled
- Circulation: the gas is moved to (and from) the tissues via a circulatory fluid
Animals typically accomplish gas exchange via one (or more) of several mechanisms and structures:
- Direct diffusion across the outer membrane is sufficient for many small organisms less than 1 mm in diameter. This process occurs in cnidarians and flatworms, where every cell in the body is close to the external environment. Their cells are kept moist, and gases diffuse quickly via direct diffusion. Flatworms are small, literally flat worms, which “breathe” through diffusion across the outer membrane. The flat shape of these organisms increases the surface area for diffusion, ensuring that each cell within the body is close to the outer membrane surface and has access to oxygen. If the flatworm had a cylindrical body, then the cells in the center would not be able to get oxygen.
- Skin is used as a respiratory organ in Annelids (such as earthworms) and Amphibians. These animals have a dense network of capillaries just below the skin that facilitates gas exchange between the external environment and the circulatory system. The respiratory surface must be kept moist in order for the gases to dissolve and diffuse across cell membranes.
- Tracheae are the respiratory organs of insects, consisting of a network of small tubes that carries oxygen to the entire body. Interestingly, insect respiration is independent of its circulatory system; therefore, the blood does not play a direct role in oxygen transport. Because the circulatory system is not used primarily to move gasses, but instead the gas passes directly to the needed tissues, the tracheal system is the most direct respiratory system for getting oxygen to respiratory sites. Insect bodies have openings, called spiracles, along the thorax and abdomen. These openings connect to the tubular network, allowing oxygen to pass into the body and regulating the diffusion of CO2 and water vapor. Air enters and leaves the tracheal system through the spiracles. Some insects can ventilate the tracheal system with body movements.
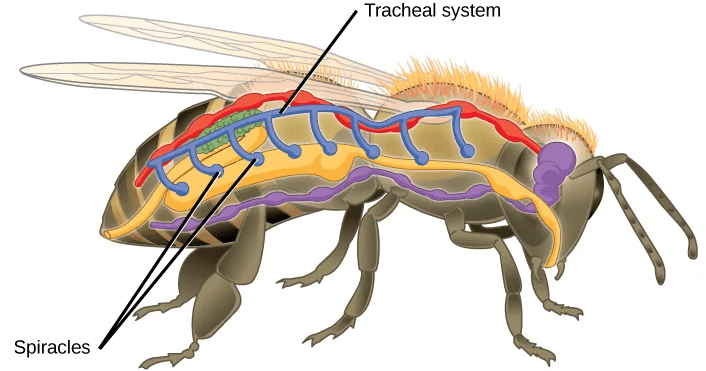
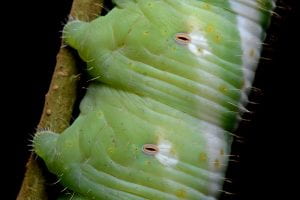
Geoff Gallice; https://commons.wikimedia.org/wiki/File:Flickr_-ggallice-_Spiracles.jpg
- Gills are defined as outgrowths of the body surface that are used for gas exchange, and they are present in organisms that live in water, including fish, mollusks, annelids, and crustaceans. Oxygen dissolves in water but at a lower concentration than in the atmosphere (much lower than the 21% oxygen that is present in the atmosphere). Gills are made of thin tissue filaments that are highly branched and folded. When water passes over the gills, the dissolved oxygen in water rapidly diffuses across the gills into the bloodstream. The circulatory system can then carry the oxygenated blood to the other parts of the body. The gills present in fish are the most efficient of all respirator surfaces due to their use of a countercurrent exchanger (also called a countercurrent amplifier) to maximize gas exchange across the length of the entire respiratory surface: because of the constant flow of gas across the gas-exchange membrane and the constant partial pressure differences, gills are the most efficient respiratory system in exchanging gases, even with the much lower concentrations of oxygen available in water compared to the atmosphere.
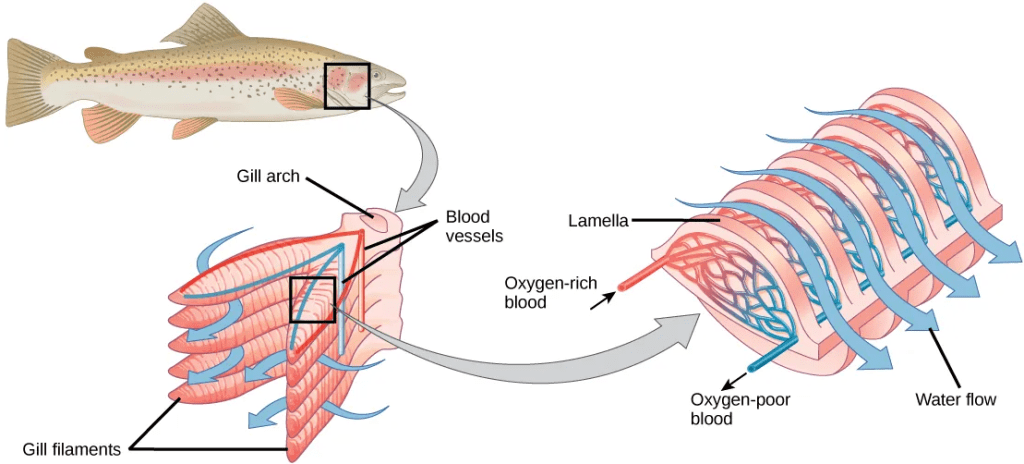
- Lungs are defined as infoldings of the throat or body surface that are used for gas exchange. Lungs are present in both invertebrate and vertebrate species, and their structure and function differs among different animal lineages:
- The lungs of amphibians supplement gas exchange that occurs via the skin. Unlike birds, mammals, and most reptiles, the lungs of amphibians are ventilated by positive pressure, where air is forced into the lungs. This process requires the amphibian to gulp air into the mouth, then close the mouth and nostrils and raise the jaw, which puts the air under high pressure and forces it to move into the lungs where the pressure is comparatively lower.
- The lungs of birds are under negative pressure, meaning that a vacuum is created by the movement of muscles in the chest which causes air to be pulled into the lungs. Avian (bird) lungs are the most efficient of all vertebrate lung systems (though they are not as efficient as fish gills), due to two adaptations not present in other lunged animals: unidirectional airflow through the lungs, and cross-current exchange between airflow and the bloodstream of the respiratory surface. Unidirectional airflow is accomplished by a series of air sacs that hold air first before and then after gas exchange has occurred, so that gas exchange occurs both during inhalation and exhalation. The cross-current exchanger helps to maintain a concentration gradient for more efficient gas exchange, though it is not nearly as efficient as countercurrent flow in fish gills. These two adaptations enable birds to obtain sufficient oxygen during flight, even at higher altitudes where the partial pressure of oxygen is much lower than at sea level.
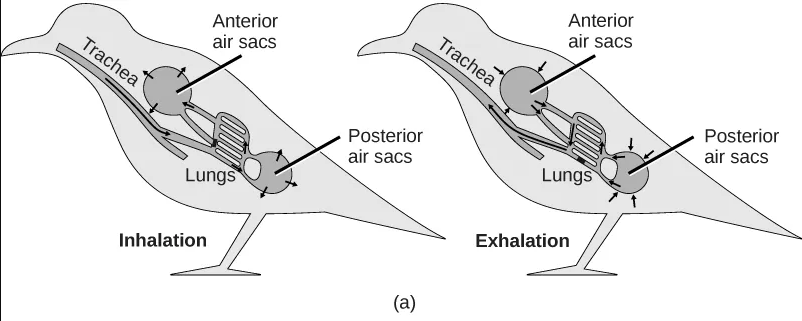
- The lungs of mammals are also under negative pressure like avian lungs. Mammalian lungs are more efficient than those of amphibians because they are under negative rather than positive pressure. However, mammalian lungs are far less efficient than those of birds: mammalian lungs lack a mechanism for unidirectional airflow, meaning gas exchange can only occur upon inhalation, and their structure does not allow for either countercurrent or cross-current gas exchange between air and blood vessels (instead relying on “web-like” flow which cannot maintain a concentration gradient). Mammalian lungs also contain a large amount of “dead space,” or locations containing inhaled air that does not take part in gas exchange. Gas exchange only occurs in small sacs called alveoli, where a web-like arrangement of blood vessels comes into close proximity to the inhaled air. The pathway to reach the alveoli includes the trachea, the bronchi, and the bronchioles, each of which are part of the “dead space” of the lungs.
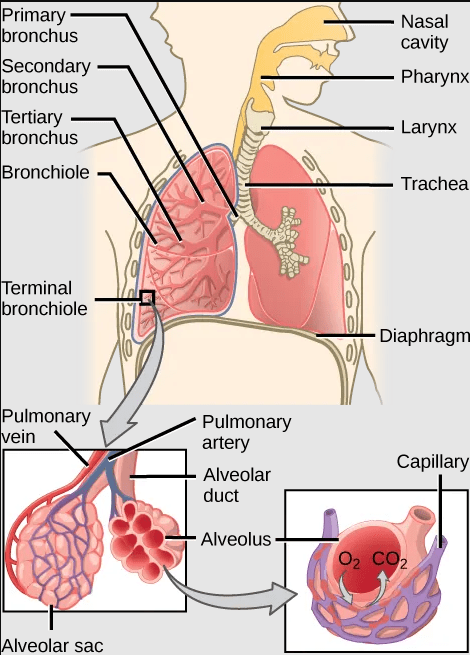
The image below shows an animated overview and comparison of the ventilation processes for mammalian lungs, avian lungs, and insect tracheae:
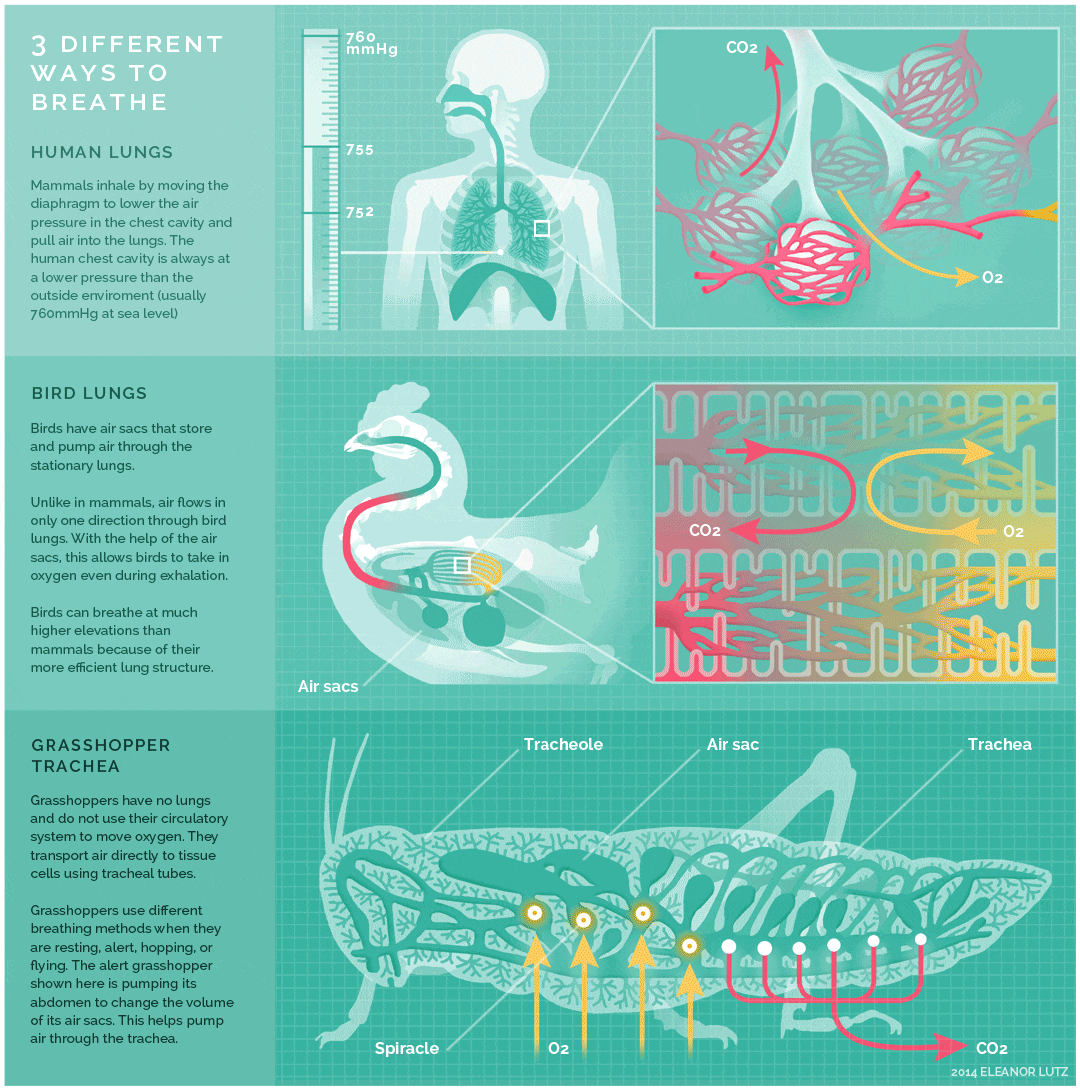
The video below provides an overview of the human respiratory system; as you watch, look for opportunities to compare and contrast with the other respiratory systems described above:
Transport of Oxygen and Carbon Dioxide in Vertebrate Respiratory Systems
The information below was adapted from OpenStax Biology 39.4
In vertebrates, blood in the circulatory system transports oxygen from the lungs to the tissues, and removes carbon dioxide from the tissue to the lungs where it is expelled from the body. Oxygen and carbon dioxide are transported by different mechanisms within the blood.
Oxygen transport: Most of the oxygen (about 98.5 percent) that enters the capillaries at the alveoli becomes bound to a protein called hemoglobin in red blood cells. (Only about 1.5 percent of oxygen in the blood is dissolved directly into the blood itself.) The hemoglobin protein is composed of four subunits, and each subunit has a central iron-containing heme group that can bind one oxygen molecule; thus each hemoglobin protein can bind to four oxygen molecules (one per subunit).

Carbon dioxide transport: Carbon dioxide molecules are transported in the blood from body tissues to the lungs by one of three methods: dissolving directly into the blood, binding to hemoglobin, or carried as a bicarbonate ion.
The majority of carbon dioxide molecules (85 percent) are carried as a bicarbonate ion, contributing to the bicarbonate buffer system. This system occurs via several sequential steps:
- Carbon dioxide diffuses into the red blood cells.
- Once in the red blood cells, the carbon dioxide is converted into carbonic acid (H2CO3).
- The unstable carbonic acid immediately dissociates into bicarbonate ions (HCO3–) and protons (H+ ions).
- Since carbon dioxide is quickly converted into bicarbonate ions, the bicarbonate buffer system allows for the continued uptake of carbon dioxide into the blood down its concentration gradient. It also results in the production of H+ ions. If too much H+ is produced, it can alter blood pH (more on this phenomenon below).
- When the blood reaches the lungs, the bicarbonate ion binds with a proton and is converted back to carbonic acid and then carbon dioxide.
- The carbon dioxide diffuses out of the blood and into the lungs, where it is expelled during exhalation.

One important benefit of the bicarbonate buffer system is that carbon dioxide is “soaked up” into the blood with little change to the pH of the overall bloodstream; this phenomenon is important because it takes only a small change in the overall pH of the body for severe injury or death to result.
Dissociation Curves Demonstrate the Cooperative Binding of Hemoglobin to Oxygen
The information below was adapted from OpenStax Biology 39.4
Hemoglobin exhibits a phenomenon called cooperative binding, where it is easier for hemoglobin to bind a second and third oxygen molecule than the first oxygen molecule. Cooperative binding occurs because the hemoglobin molecule changes its shape, or conformation, after the first oxygen binds; the resulting change in shape increases the ability of the next oxygen to bind to hemoglobin and achieve greater oxygen saturation.
We can plot the binding of oxygen to hemoglobin as a function of the partial pressure of oxygen in the blood (x-axis) versus the relative hemoglobin-oxygen saturation (y-axis). The resulting graph, an oxygen dissociation curve, is sigmoidal, or S-shaped. The S-shape illustrates cooperative binding in action: as the partial pressure of oxygen increases, the hemoglobin becomes increasingly saturated with oxygen.
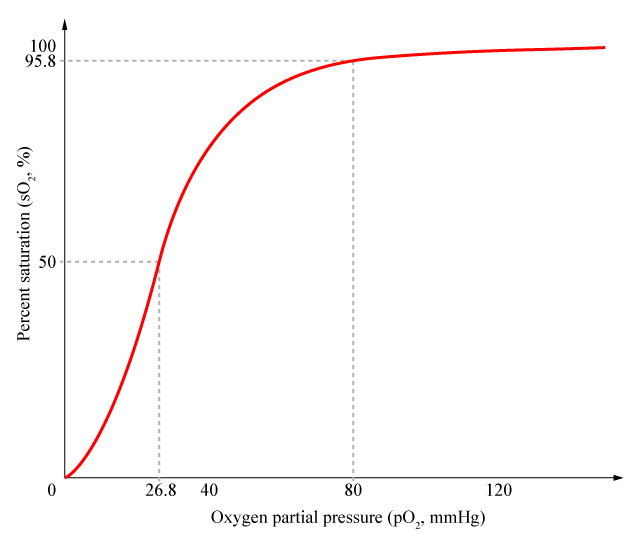
Factors That Affect the Hemoglobin Dissociation Curve for Oxygen Binding
The information below was adapted from OpenStax Biology 39.4
The oxygen-carrying capacity of hemoglobin determines how much oxygen is carried in the blood. In addition to the partial pressure of oxygen, other factors that can affect oxygen carrying capacity and delivery include pH, temperature, and carbon dioxide concentration.
As described above, carbon dioxide in the blood is converted to bicarbonate (HCO3-) and protons (H+). As the level of carbon dioxide in the blood increases, more H+ is produced and thus blood pH decreases. The decrease in pH (increase in acidity) reduces the affinity of hemoglobin for oxygen. As a result, oxygen dissociates more easily from hemoglobin, shifting the oxygen dissociation curve to the right (and thus more readily offloading oxygen to tissues).
A similar shift in the curve also results from an increase in body temperature: increased temperature, such as from increased activity of skeletal muscle, causes the affinity of hemoglobin for oxygen to be reduced such that hemoglobin more easily releases oxygen to tissues.
Use the graph below to predict how easily hemoglobin releases oxygen to tissues when pH is low (carbon dioxide levels are high) compared to when pH is high (carbon dioxide levels are low):
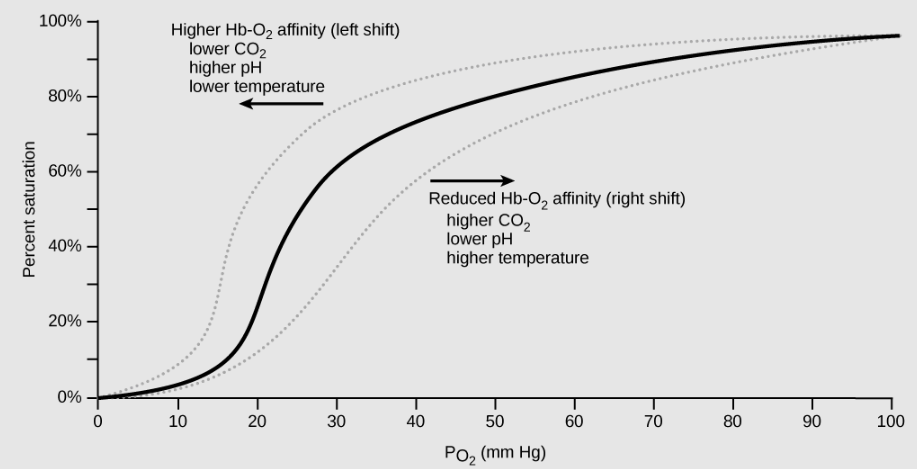
The video below provides an overview of the transport of oxygen and carbon dioxide in the human bloodstream: